The diagnostic role of fat in osteosarcopenia
Introduction
Sarcopenia (loss of muscle mass and strength) and osteoporosis (bone loss) are two of the most prevalent chronic diseases in old age. When occurring simultaneously, a syndrome known as osteosarcopenia (1,2), the incidence of negative outcomes such as falls, fractures, loss of function, frailty, and mortality increases (3-5).
The diagnosis of osteosarcopenia is challenging. Lack of unified diagnostic criteria for sarcopenia (6), considerable disagreement between sarcopenia diagnostic algorithms (7), and the limitations of bone mineral density (BMD) as the gold standard for the diagnosis of osteopenia and osteoporosis (8,9) have limited the capacity of the clinician to appropriately identify and treat this syndrome. Therefore, more accurate and valid diagnostic methods that either complement or replace current clinical and paraclinical methods are in demand.
Fat infiltration is one of the hallmarks of sarcopenia and osteoporosis (10,11), thus of osteosarcopenia (3). High levels of marrow adipose tissue (MAT) are associated with bone loss and osteoporosis (11). MAT secretes adipocytokines and fatty acids, which are toxic to the cells in the vicinity of adipocytes, decreasing bone formation and increasing bone resorption (12,13). Similarly, fat infiltration in muscle fibers is associated with cell dysfunction (14).
In this review, we will discuss and summarize the salient developments in the current understanding of the role of fat infiltration in osteosarcopenia, and the potential use of fat as a biomarker for this syndrome.
Osteosarcopenia as a fat-related disease
The progressive and synchronic infiltration of fat in muscle and bone marrow in sarcopenia and osteoporosis, respectively, is suggestive of a shared mechanism (3). Bone, muscle and fat are all derived from the embryonic mesoderm. In addition, marrow and muscle adipocytes share the same type of mesenchymal stem cell (MSC) precursors. However, the distribution of those MSC differ for bone and muscle. In the bone marrow milieu, MSC develop perivascular niches, which act as an epicentre of cross-talk between cell lines and as a supplier of MSC for osteogenesis (15). Under the appropriate conditions, perivascular cells possess the ability to differentiate into MSC lineages, including chondrocytes, multilocular adipocytes, and osteoblasts (15). With aging, the capacity of MSC to differentiate into chondrocytes and osteoblasts is reduced and followed by an increase in their differentiation into adipocytes, thus affecting bone formation (16). This is associated with other age-related changes in the MSC niche such as oxidative stress, changes in hormones and growth factors, and “chaotic” cell-cell cross-talking (16).
Increasing number and size of marrow adipocytes are associated with the secretion of higher concentrations of adipocytokines and fatty acids within the marrow milieu (12). Our previous experiments using co-cultures of adipocytes and osteoblasts demonstrated that the fatty acids (predominantly palmitic acid) produced by marrow adipocytes hinder osteoblastogenesis, bone formation and cell survival in vitro (17). When exposed to palmitate in concentrations similar to those observed in bone marrow (18), human osteoblasts reduce their capacity to differentiate from pre-osteoblast stage, show dysfunctional osteogenic pathways (19), and undergo apoptosis and dysfunctional autophagy (20). Overall, although the lipotoxic effects of marrow adipocytes have been well documented in vitro (17,19-21), this direct association is yet to be documented in vivo. Nevertheless, human studies have reported a clear inverse correlation between MAT and bone mass (13,22), which is independent of BMI, suggesting that the bone loss observed in these subjects was associated with local, rather than systemic factors. Also, in a dog model, it has been shown that, compared to the bone facing red marrow, bone apposition rate and formation surface ratio are reduced in trabecular bone adjacent to fatty marrow (23).
In the muscle, intermuscular adipose tissue (IMAT) (24), which is a depot of adipocytes located between muscle bundles, and lipid infiltration within myofibers, known as intramuscular fat or intramyocellular (IMC) lipid, are increased in aging and sarcopenia. Increased IMAT and IMC are both associated with insulin insensitivity, inflammation, and functional deficits in skeletal muscle (25-28). This infiltration is explained by several mechanisms, including de-differentiation of muscle-derived stem cells or other mesenchymal progenitors into cells with adipocyte phenotype. Amongst those stem cells, muscle satellite cells—a heterogeneous stem cell population characterized by plasticity and self-renewal that allow muscular growth and regeneration—can acquire features of adipocytes. Similar to bone marrow MSC failure to express the transcription factors that direct mesenchymal precursors into fully differentiated functionally specialized cells, with increasing lipotoxicity, satellite cells may also be driven to switch their phenotype into the adipogenic lineage. Similar to MAT, IMAT and IMC adipocytes secrete lipotoxic adipocytokines and fatty acids that affect myocyte function by: (I) decreasing insulin sensitivity and impairing the capacity for normal protein synthesis in skeletal muscle; (II) supporting a transition of type II fibers to a type I phenotype; and (III) inducing loss of muscle fibers and replacement with fatty and fibrous tissues (3,24-28). As with MAT, increasing levels of IMAT and IMC in muscle are also independent of BMI; suggesting an age-related local mechanism, which could be aggravated by systemic factors such as corticosteroids, vitamin D levels, leptin and sex steroid deficiency (29).
Interestingly, the pathways that have been implicated in marrow and muscle adipogenesis are almost identical. Pathways and factors such as Peroxisome proliferator-activated receptors (PPARs), WNTs, growth factors, myokines, osteokines, guanine nucleotide exchange factors (GEF)-GAP-Rho, p66, mitochondrial ROS production, and protein kinase C beta (PKCβ) are common to bone and muscle and could be implicated in the adipogenic conversion of MSC and satellite cells (3,25,27). Targeting these factors to prevent accumulation of fat in these tissues is a subject of intensive research.
Indeed, the potential effect of lipotoxicity is increasingly identified in various other aging-associated conditions. Decline in lymphopoiesis coincides with fat infiltration into marrow and lymphoid organs (30,31). Recently, MAT accumulation in lymph nodes, mammary glands and intestine was shown to be associated with the occurrence of leukemias (32), postmenopausal breast cancer risk (33) and colorectal adenoma (34), respectively. Local lipotoxicity and inflammation due to production of high concentrations of inflammatory mediators and fatty acids (of which minimal amounts are secreted into the circulation) (35) do induce malignancies (36). Therefore, the reported atrophy of red marrow with age-associated MAT expansion (37) is potentially capable of inducing both anaplasia and immunodeficiency. Some possible molecular pathogenesis mechanisms by which ectopic fat infiltration causes inflammation and anaplasia have been described (38).
In addition, high dietary inflammatory index (39) is associated with the development of sarcopenia (40) as well as osteoporosis and risk of fractures (41). It has also been theoretically linked to osteosarcopenia, particularly in obese people (42). Nevertheless, considering the close associations of the index with risk factors for obesity and ectopic fat infiltration and lipotoxicity, it is likely that an inflammatory diet [high in carbohydrates, meat and trans fatty acids and low in fibre and vegetables (43)] can predispose subjects to a variety of fat-related conditions including osteosarcopenia.
Fat quantification as a diagnostic method for osteosarcopenia
Bone and muscle fat: a systemic role
In bone, fat infiltration is observed since early age. Bone accrual in the appendicular skeleton of healthy young adults is inversely related to changes in marrow adiposity (44,45). Regarding muscle, IMAT and IMC infiltration is accelerated after the 5th decade; however, younger individuals—especially those sedentary or with risk factors such as diabetes—show some levels of fat infiltration as well (46).
The pathophysiological significance of bone and muscle fat infiltration goes beyond the local bone marrow and muscle milieu. However, marrow and muscle adipose tissue behave somewhat differently than other types of fat such as visceral (VAT) and subcutaneous adipose tissue (SAT). In a study in 131 healthy young adults (60 females, 71 males; 16–25 years of age), Di Giorgi et al. (47) reported that marrow adiposity in young men and women is independent of VAT and SAT and is not associated with cardiovascular risk; therefore, supporting the concept that marrow adiposity is not involved in the comorbidities related to fat accumulation in other compartments. We compared VAT, SAT and MAT in healthy older men (mean age 67±5.5) and investigated their serum biochemistry, and inflammatory panel (37). Our results confirmed strong inverse associations between MAT and bone mass and volume and red marrow volume. However, MAT volume in all regions of interest was correlated, and unlike reports in women (48-50), mainly independent of VAT or SAT volumes. Overall, the discordant associations of MAT with circulating metabolic and inflammatory indices provided additional indication on MAT's distinct attributes compared to VAT and SAT.
Due to the major metabolic role of muscle, fat infiltration is expected to induce metabolic abnormalities, which are partly dissimilar to MAT. Accumulation of muscle fat decreases insulin sensitivity, and as muscles are one of the major consumers of glucose, such insensitivity could lead to increased blood glucose and diabetes (51). In addition, we recently reported that muscle loss and high levels of fat infiltration and atrophy of certain lower limb muscles in levator ani in older men receiving androgen deprivation therapy may contribute to both sarcopenia and sexual dysfunction in these subjects (52).
Overall, very few studies have looked at the systemic effect of IMAT and IMC, probably due to the technical challenges to accurately quantify these types of fat, most particularly IMC.
Fat products as biomarkers for osteosarcopenia
MAT and muscle fat (IMAT and IMC) have not been fully characterised. All express characteristics of brown and white fat—a phenotype that has been denominated as “beige” by some teams (53-56). Having a beige phenotype means that MAT, IMAT and IMC adipocytes have a combination of the thermogenic and anabolic function of brown adipocytes combined with the metabolic and potential lipotoxic function of white adipocytes (12,53-56). In addition, by having this very specific phenotype, it would be expected that MAT, IMAT, and IMC adipocytes secrete a particular set of adipokines and other factors that could reach the circulation, and then they could be used as biomarkers for osteoporosis and sarcopenia.
We tested this hypothesis by characterizing the age-related changes in cytokine expression by MAT as compared with SAT (57). MAT and SAT adipocytes were isolated from young (4 months) and old (24 months) males C57BL/6J, and proteomic analysis of 96 cytokines was performed using a cytokine antibody array. Proteins that were grouped according with their known function in bone showed a substantial change. We found a significant age-induced difference in the expression of 53 cytokines. As compared with SAT adipocytes, aging MAT adipocytes showed a more pro-adipogenic, anti-osteoblastogenic and pro-apoptotic phenotype. Overall, our data suggested that, with aging, MAT adipocytes become significantly more toxic than SAT adipocytes. We concluded that these cytokines, if secreted into the intercellular space, could play a role in the pathogenesis of age-related bone loss by affecting other cells within the marrow milieu. Interestingly, several cytokines were highly expressed in aged MAT, particularly matrix metalloproteinase-2 (MMP-2) and resistin. Although these cytokines are not exclusive to MAT, increasing levels could be used as an indicator of high levels of marrow fat and possibly a predictor of osteoporotic fractures in older persons. However, further studies are still required.
In muscle, adipocytokines have usually been associated with systemic regulators of muscle formation and metabolism predominantly adiponectin and leptin (58). However, the association between adipocytokines and sarcopenia has focused on the concept of sarcopenic obesity, which is associated with high levels of adipokines usually observed in obese individuals (58,59). Regarding specific analyses of adipocyte-secreted products of IMAT and IMC, Zoico et al. (60) performed biopsies of paraspinatus muscle of 16 older men undergoing elective vertebral surgery and histologically determined IMAT and quantified gene expression in IMAT and in SAT for IL-6, C-reactive protein (CRP), vascular endothelial growth factor (VEGF) and adiponectin. As expected, leptin was positively associated with both types of fat, whereas CRP was only associated with IMAT. As per our knowledge, no other studies have investigated the specific characteristics and secretory profile of IMAT or IMC.
In summary, further studies aimed to identify a particular (and maybe concurrent) secretory profile of MAT, IMAT and IMC, which seems to be independent of other types of fat and/or obesity, are still required. Identification of a common adipocyte-secreted adipocytokine(s) for these three types of fat would have a strong potential to become robust biomarkers for osteosarcopenia in the future.
Fat imaging in osteosarcopenia
In order to advance our understanding of the role of fat infiltration in osteosarcopenia, it will be necessary to develop, test, and validate novel and accurate imaging methods capable of quantifying adiposity within bone marrow and muscle fibers and ultimately assess its functionality. In addition, newer techniques capable of imaging fat in muscle and bone may provide additional diagnostic and prognostic utility and may be useful in guiding therapeutic interventions.
Dual-energy X-ray Absorptiometry (DXA) uses low level X-rays providing estimates of fat mass, fat-free mass, and bone density (61). However, DXA has major limitations to be considered as the optimal imaging diagnostic method for osteosarcopenia. It is unable to identify and quantify MAT, IMAT and IMC and it only quantifies lean mass, which considering that no one technique subserves all requirements to accurately quantify muscle mass, has been proposed as a reference standard (but not a gold standard) for measuring muscle lean body mass (62).
Computed tomography (CT) is another non-invasive method that determines the cross-sectional distribution of the X-ray absorption coefficient, which after normalization to the absorption of air and water is called CT value and measured in Hounsfield units (HU). CT slices of predefined width can be analysed for different tissues, using manual segmentation or automated software. For example, muscle area, or in case of the analysis of a stack of images, volume of individual muscles, or a group of muscles can be determined. By definition, the HU value of air is −1,000 and of water 0. Bone, skeletal muscle, adipose tissue, and visceral organs have specific Hounsfield unit ranges, allowing for their quantification in the images. Our team has validated the use of a CT image analysis software to quantify MAT and IMAT (37,52,63). Using this innovative method, we quantified fat infiltration of muscle and bone of lamin A/C knock out mice (Figure 1), allowing us to propose this as a mouse model of osteosarcopenia (64). However, compared to DXA, CT has several limitations including a higher cost and radiation dose, which limit the regular use of CT in clinical practice. In addition, due to its low resolution, CT cannot be used to quantify IMC, and possibly underestimates marrow fat due to beam hardening artefacts.
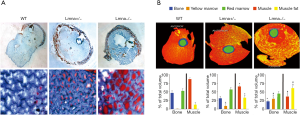
Peripheral quantitative CT (pQCT) and high resolution pQCT are an alternative to CT with capacity to quantify muscle, bone, MAT and IMAT. We used this technique to determine the characteristics of sarcopenic obese older individuals that are independently associated with bone health and balance (65). Using this technique, we also reported that calf muscle density (which is inversely related to infiltrated fat volume) is independently associated with physical function in overweight and obese older adults (66). pQCT benefits from its portability, unrestricted gantry depth and therefore ability to scan sites as proximal as the thigh. While high resolution pQCT is in the early development stages of making muscle measurement possible, it already excels in quantifying bone architecture by virtue of its ability to image using smaller voxel sizes and a larger image stack compared to pQCT (67). However, limitations of these methods include cost, sensitivity to patient motion, and lack of reference values and standards. In addition, their ability in quantifying marrow fat is yet to be validated.
Magnetic resonance imaging (MRI) is also a useful imaging method in osteosarcopenia because, by measuring differences in spin relaxation properties of protons in different chemical environments, MRI can generate images with higher contrast between soft tissues and bone than QCT (67), generating much higher contrast between muscle and inter/intramuscular fat and MAT than CT-based modalities. Another major advantage is the fact that MRI confers no ionizing radiation exposure. Disadvantages of this method include cost, lack of portability, and limited ability to quantify bone mineral density.
As an alternative to MRI, peripheral MRI (pMRI) has advantages similar to MRI in terms of capacity to differentiate and quantify bone, muscle and fat (including IMAT), occupying a small footprint compared to full body scanners. Although the sensitivity of this technique to quantify MAT and IMAT has improved in recent years, no study has used pMRI to diagnose osteosarcopenia in human subjects. Development of affordable systems that can carry out nuclear magnetic resonance spectroscopy, which can provide detailed composition data on infiltrated fatty tissue and its volume in bone and muscle, would be of great diagnostic value for osteosarcopenia in both clinical and research settings.
Overall, imaging techniques could play an important role in the diagnosis of osteosarcopenia and in the prediction of its adverse outcomes and response to current and future treatments. A major limitation of these methods is their lack of capacity to quantify IMC fat, which presently can only be quantified by staining muscle biopsies with oil red O. However, with improving resolution of MRI and QCT images, quantifying IMC with these methods could be a feasible possibility in the near future.
Conclusions
Osteosarcopenia is a new geriatric syndrome that increases the risk for falls, fractures, disability, frailty and early mortality in our older population (68). Therefore, early identification of this syndrome and accurate prediction of poor outcomes and response to treatment are crucial. Sarcopenia and osteoporosis are two sides of the same coin sharing similar risk factors and pathophysiology (69) in which fat is a major player (3). Apart of synchronic bone and muscle loss in osteosarcopenia, fat infiltration is the most constant finding in both diseases, therefore it is the most natural diagnostic and therapeutic target. As secretory tissues, MAT, IMAT and IMC release adipocytokines that could be quantified in the circulation, however, identification of these specific markers is still in a very early phase. On the other hand, imaging methods offer a promissory approach by increasing their resolution to identify muscle, bone, MAT and IMAT thus increasing their potential to be used in the diagnosis of osteosarcopenia, once the appropriate standards have been determined.
Acknowledgments
Funding: None.
Footnote
Provenance and Peer Review: This article was commissioned by the Guest Editors (Markus Herrmann and Barbara Obermayer-Pietsch) for the series “Clinical and Analytical Aspects of Bone and Intersystemic Diseases” published in Journal of Laboratory and Precision Medicine. The article has undergone external peer review.
Conflicts of Interest: All authors have completed the ICMJE uniform disclosure form (available at http://dx.doi.org/10.21037/jlpm.2019.02.01). The series “Clinical and Analytical Aspects of Bone and Intersystemic Diseases” was commissioned by the editorial office without any funding or sponsorship. The authors have no other conflicts of interest to declare.
Ethical Statement: The authors are accountable for all aspects of the work in ensuring that questions related to the accuracy or integrity of any part of the work are appropriately investigated and resolved.
Open Access Statement: This is an Open Access article distributed in accordance with the Creative Commons Attribution-NonCommercial-NoDerivs 4.0 International License (CC BY-NC-ND 4.0), which permits the non-commercial replication and distribution of the article with the strict proviso that no changes or edits are made and the original work is properly cited (including links to both the formal publication through the relevant DOI and the license). See: https://creativecommons.org/licenses/by-nc-nd/4.0/.
References
- Zanker J, Duque G. Osteoporosis in Older Persons: Old and New Players. J Am Geriatr Soc 2018; [Epub ahead of print]. [Crossref] [PubMed]
- Drey M, Sieber CC, Bertsch T, et al. Osteosarcopenia is more than sarcopenia and osteopenia alone. Aging Clin Exp Res 2016;28:895-9. [Crossref] [PubMed]
- Hirschfeld HP, Kinsella R, Duque G. Osteosarcopenia: where bone, muscle, and fat collide. Osteoporos Int 2017;28:2781-90. [Crossref] [PubMed]
- Yoo JI, Kim H, Ha YC, et al. Osteosarcopenia in Patients with Hip Fracture Is Related with High Mortality. J Korean Med Sci 2018;33:e27 [Crossref] [PubMed]
- Pasco JA, Mohebbi M, Holloway KL, et al. Musculoskeletal decline and mortality: prospective data from the Geelong Osteoporosis Study. J Cachexia Sarcopenia Muscle 2017;8:482-9. [Crossref] [PubMed]
- Han A, Bokshan SL, Marcaccio SE, et al. Diagnostic Criteria and Clinical Outcomes in Sarcopenia Research: A Literature Review. J Clin Med 2018;7. [PubMed]
- Reijnierse EM, Trappenburg MC, Leter MJ, et al. The Impact of Different Diagnostic Criteria on the Prevalence of Sarcopenia in Healthy Elderly Participants and Geriatric Outpatients. Gerontology 2015;61:491-6. [Crossref] [PubMed]
- Choksi P, Jepsen KJ, Clines GA. The challenges of diagnosing osteoporosis and the limitations of currently available tools. Clin Diabetes Endocrinol 2018;4:12. [Crossref] [PubMed]
- Marshall D, Johnell O, Wedel H. Meta-analysis of how well measures of bone mineral density predict occurrence of osteoporotic fractures. BMJ 1996;312:1254-9. [Crossref] [PubMed]
- Ji HM, Han J, Won YY. Sarcopenia and Osteoporosis. Hip Pelvis 2015;27:72-6. [Crossref] [PubMed]
- Hardouin P, Rharass T, Lucas S. Bone Marrow Adipose Tissue: To Be or Not To Be a Typical Adipose Tissue? Front Endocrinol (Lausanne) 2016;7:85. [Crossref] [PubMed]
- Singh L, Tyagi S, Myers D, et al. Good, Bad, or Ugly: the Biological Roles of Bone Marrow Fat. Curr Osteoporos Rep 2018;16:130-7. [Crossref] [PubMed]
- Verma S, Rajaratnam JH, Denton J, et al. Adipocytic proportion of bone marrow is inversely related to bone formation in osteoporosis. J Clin Pathol 2002;55:693-8. [Crossref] [PubMed]
- Schaap LA, Koster A, Visser M. Adiposity, muscle mass, and muscle strength in relation to functional decline in older persons. Epidemiol Rev 2013;35:51-65. [Crossref] [PubMed]
- Oh M, Nör JE. The Perivascular Niche and Self-Renewal of Stem Cells. Front Physiol 2015;6:367. [Crossref] [PubMed]
- Ganguly P, El-Jawhari JJ, Giannoudis PV, et al. Age-related Changes in Bone Marrow Mesenchymal Stromal Cells: A Potential Impact on Osteoporosis and Osteoarthritis Development. Cell Transplant 2017;26:1520-9. [Crossref] [PubMed]
- Elbaz A, Wu X, Rivas D, Gimble JM, et al. Inhibition of fatty acid biosynthesis prevents adipocyte lipotoxicity on human osteoblasts in vitro. J Cell Mol Med 2010;14:982-91. [Crossref] [PubMed]
- Griffith JF, Yeung DK, Ahuja AT, et al. A study of bone marrow and subcutaneous fatty acid composition in subjects of varying bone mineral density. Bone 2009;44:1092-6. [Crossref] [PubMed]
- Gunaratnam K, Vidal C, Gimble JM, et al. Mechanisms of palmitate-induced lipotoxicity in human osteoblasts. Endocrinology 2014;155:108-16. [Crossref] [PubMed]
- Gunaratnam K, Vidal C, Boadle R, et al. Mechanisms of palmitate-induced cell death in human osteoblasts. Biol Open 2013;2:1382-9. [Crossref] [PubMed]
- Kim JE, Ahn M-W, Baek S-H, et al. AMPK activator, AICAR, inhibits palmitate-induced apoptosis in osteoblast. Bone 2008;43:394-404. [Crossref] [PubMed]
- Kim DH, Lim H, Chang S, et al. Association between Body Fat and Bone Mineral Density in Normal-Weight Middle-Aged Koreans. Korean J Fam Med 2018; [Epub ahead of print]. [Crossref] [PubMed]
- Wronski TJ, Smith J, Jee W. Variations in mineral apposition rate of trabecular bone within the beagle skeleton. Calcif. Tissue Int 1981;33:583. [Crossref] [PubMed]
- Vettor R, Milan G, Franzin C, et al. The origin of intermuscular adipose tissue and its pathophysiological implications. Am J Physiol Endocrinol Metab 2009;297:E987-8. [Crossref] [PubMed]
- Hamrick MW, McGee-Lawrence ME, Frechette DM. Fatty Infiltration of Skeletal Muscle: Mechanisms and Comparisons with Bone Marrow Adiposity. Front Endocrinol (Lausanne) 2016;7:69. [Crossref] [PubMed]
- Komolka K, Albrecht E, Wimmers K, et al. Molecular heterogeneities of adipose depots – potential effects on adipose-muscle cross-talk in humans, mice and farm animals. J Genomics 2014;2:31-44. [Crossref] [PubMed]
- Kindler JM, Lewis RD, Hamrick MW. Skeletal muscle and pediatric bone development. Curr Opin Endocrinol Diabetes Obes 2015;22:467-74. [Crossref] [PubMed]
- Rivas DA, McDonald DJ, Rice NP, et al. Diminished anabolic signaling response to insulin induced by intramuscular lipid accumulation is associated with inflammation in aging but not obesity. Am J Physiol Regul Integr Comp Physiol 2016;310:R561-9. [Crossref] [PubMed]
- Takano Y, Kobayashi H, Yuri T, et al. Fat infiltration in the gluteus minimus muscle in older adults. Clin Interv Aging 2018;13:1011-7. [Crossref] [PubMed]
- Kennedy DE, Witte PL, Knight KL. Bone marrow fat and the decline of B lymphopoiesis in rabbits. Dev Comp Immunol 2016;58:30-9. [Crossref] [PubMed]
- Hadamitzky C, Spohr H, Debertin AS, et al. Age-dependent histoarchitectural changes in human lymph nodes: an underestimated process with clinical relevance? J Anat. 2010;216:556-62. [Crossref] [PubMed]
- Karaosmanoglu AD, Blake MA, Lennerz JK. Abundant macroscopic fat in intra-abdominal lymph nodes involved in the course of a patient with chronic lymphocytic leukaemia: presentation of imaging findings with biopsy correlation. Br J Radiol 2012;85:e91-3. [Crossref] [PubMed]
- Li G, Xu Z, Zhuang A, et al. Magnetic Resonance Spectroscopy-Detected Change in Marrow Adiposity Is Strongly Correlated to Postmenopausal Breast Cancer Risk. Clin Breast Cancer 2017;17:239-44. [Crossref] [PubMed]
- Xu H, Zhang Y, Dong H, et al. To assess the association between vertebral marrow fat content and colorectal adenoma in postmenopausal women using magnetic resonance spectroscopy. Acta Radiol 2016;57:1033-9. [Crossref] [PubMed]
- Coppack SW. Pro-inflammatory cytokines and adipose tissue. Proc Nutr Soc 2001;60:349-56. [Crossref] [PubMed]
- Balkwill F, Mantovani A. Inflammation and cancer: back to Virchow? Lancet 2001;357:539-45. [Crossref] [PubMed]
- Bani Hassan E, Demontiero O, Vogrin S, et al. Marrow Adipose Tissue in Older Men: Association with Visceral and Subcutaneous Fat, Bone Volume, Metabolism, and Inflammation. Calcif Tissue Int 2018;103:164-74. [Crossref] [PubMed]
- Khandekar MJ, Cohen P, Spiegelman BM. Molecular mechanisms of cancer development in obesity. Nat Rev Cancer 2011;11:886. [Crossref] [PubMed]
- Shivappa N, Steck SE, Hurley TG, et al. Designing and developing a literature-derived, population-based dietary inflammatory index. Public Health Nutr 2014;17:1689-96. [Crossref] [PubMed]
- Park S, Na W, Sohn C. Relationship between osteosarcopenic obesity and dietary inflammatory index in postmenopausal Korean women: 2009 to 2011 Korea National Health and Nutrition Examination Surveys. J Clin Biochem Nutr 2018;63:211-6. [Crossref] [PubMed]
- Kim HS, Sohn C, Kwon M, et al. Positive Association between Dietary Inflammatory Index and the Risk of Osteoporosis: Results from the KoGES_Health Examinee (HEXA) Cohort Study. Nutrients 2018;10. [PubMed]
- Stefanaki C, Pervanidou P, Boschiero D, et al. Chronic stress and body composition disorders: implications for health and disease. Hormones (Athens) 2018;17:33-43. [Crossref] [PubMed]
- Bulló M, Casas-Agustench P, Amigó-Correig P, Aranceta J, Salas-Salvadó J. Inflammation, obesity and comorbidities: the role of diet. Public Health Nutr 2007;10:1164-72. [Crossref] [PubMed]
- Di Iorgi N, Rosol M, Mittelman SD, et al. Reciprocal relation between marrow adiposity and the amount of bone in the axial and appendicular skeleton of young adults. J Clin Endocrinol Metab 2008;93:2281-6. [Crossref] [PubMed]
- Di Iorgi N, Mo AO, Grimm K, et al. Bone acquisition in healthy young females is reciprocally related to marrow adiposity. J Clin Endocrinol Metab. 2010;95:2977-82. [Crossref] [PubMed]
- Marcus RL, Addison O, Kidde JP, et al. Skeletal muscle fat infiltration: impact of age, inactivity, and exercise. J Nutr Health Aging 2010;14:362-6. [Crossref] [PubMed]
- Di Iorgi N, Mittelman SD, Gilsanz V. Differential effect of marrow adiposity and visceral and subcutaneous fat on cardiovascular risk in young, healthy adults. Int J Obes (Lond) 2008;32:1854-60. [Crossref] [PubMed]
- Baum T, Yap SP, Karampinos DC, et al. Does vertebral bone marrow fat content correlate with abdominal adipose tissue, lumbar spine BMD and blood biomarkers in women with type 2 diabetes mellitus? J Magn Reson Imaging 2012;35:117-24. [Crossref] [PubMed]
- Bredella MA, Torriani M, Ghomi RH, et al. Vertebral Bone Marrow Fat Is Positively Associated With Visceral Fat and Inversely Associated With IGF-1 in Obese Women. Obesity (Silver Spring) 2011;19:49-53. [Crossref] [PubMed]
- L Newton A. The relationships among total body fat, bone mineral content and bone marrow adipose tissue in early-pubertal girls. Bonekey Rep 2013;2:315. [PubMed]
- Scott D, de Courten B, Ebeling PR. Sarcopenia: a potential cause and consequence of type 2 diabetes in Australia's ageing population? Med J Aust 2016;205:329-33. [Crossref] [PubMed]
- Cheung AS, Cunningham C, Ko DKD, et al. Selective loss of levator ani and leg muscle volumes in men undergoing androgen deprivation therapy. J Clin Endocrinol Metab 2018; [Epub ahead of print]. [Crossref] [PubMed]
- Bukowska J, Frazier T, Smith S, et al. Bone Marrow Adipocyte Developmental Origin and Biology. Curr Osteoporos Rep 2018;16:312-9. [Crossref] [PubMed]
- Horowitz MC, Berry R, Holtrup B, et al. Bone marrow adipocytes. Adipocyte 2017;6:193-204. [Crossref] [PubMed]
- Rui L. Brown and Beige Adipose Tissues in Health and Disease. Compr Physiol 2017;7:1281-306. [Crossref] [PubMed]
- Wang W, Seale P. Control of brown and beige fat development. Nat Rev Mol Cell Biol 2016;17:691-702. [Crossref] [PubMed]
- Gasparrini M, Rivas D, Elbaz A, et al. Differential expression of cytokines in subcutaneous and marrow fat of aging C57BL/6J mice. Exp Gerontol 2009;44:613-8. [Crossref] [PubMed]
- Polyzos SA, Margioris AN. Sarcopenic obesity. Hormones (Athens) 2018;17:321-31. [Crossref] [PubMed]
- Ilich JZ, Kelly OJ, Inglis JE, et al. Interrelationship among muscle, fat, and bone: connecting the dots on cellular, hormonal, and whole-body levels. Ageing Res Rev 2014;15:51-60. [Crossref] [PubMed]
- Zoico E, Corzato F, Bambace C, et al. Myosteatosis and myofibrosis: relationship with aging, inflammation and insulin resistance. Arch Gerontol Geriatr 2013;57:411-6. [Crossref] [PubMed]
- Wang H, Chen YE, Eitzman DT. Imaging body fat: techniques and cardiometabolic implications. Arterioscler Thromb Vasc Biol 2014;34:2217-23. [Crossref] [PubMed]
- Buckinx F, Landi F, Cesari M, et al. Pitfalls in the measurement of muscle mass: a need for a reference standard. J Cachexia Sarcopenia Muscle 2018;9:269-78. [Crossref] [PubMed]
- Demontiero O, Li W, Thembani E, et al. Validation of noninvasive quantification of bone marrow fat volume with microCT in aging rats. Exp Gerontol 2011;46:435-40. [Crossref] [PubMed]
- Tong J, Li W, Vidal C, et al. Lamin A/C deficiency is associated with fat infiltration of muscle and bone. Mech Ageing Dev 2011;132:552-9. [Crossref] [PubMed]
- Scott D, Shore-Lorenti C, McMillan L, et al. Associations of components of sarcopenic obesity with bone health and balance in older adults. Arch Gerontol Geriatr 2018;75:125-31. [Crossref] [PubMed]
- Scott D, Shore-Lorenti C, McMillan LB, et al. Calf muscle density is independently associated with physical function in overweight and obese older adults. J Musculoskelet Neuronal Interact 2018;18:9-17. [PubMed]
- Wong AK. A comparison of peripheral imaging technologies for bone and muscle quantification: a technical review of image acquisition. J Musculoskelet Neuronal Interact 2016;16:265-82. [PubMed]
- Hassan EB, Duque G. Osteosarcopenia: A new geriatric syndrome. Aust Fam Physician 2017;46:849-853. [PubMed]
- Girgis CM. Integrated therapies for osteoporosis and sarcopenia: from signaling pathways to clinical trials. Calcif Tissue Int 2015;96:243-55. [Crossref] [PubMed]
Cite this article as: Al Saedi A, Bani Hassan E, Duque G. The diagnostic role of fat in osteosarcopenia. J Lab Precis Med 2019;4:7.